Present and future applications of superconductivity: Particle accelerators, medical applications and beyond
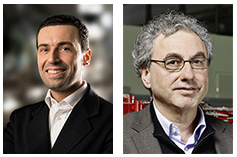
By Prof. Carmine Senatore, UNIGE and Prof. Leonid Rivkin, PSI
Since the discovery of superconductivity in 1911, this phenomenon was greeted with high hopes for groundbreaking applications. However, scientists had to face many difficulties to develop these materials in a useful conductor form and yet only six – one alloy, two intermetallic compounds and three ceramic oxides – are available commercially. After more than a century, superconducting technologies are a proven business worldwide, contributing over 7 BCHF per year to medical and high energy physics industries, but there is a potential for several times this amount for power applications in the clean energy field.
Medical and biopharma applications
Currently, the major commercial applications of superconductivity involve low-temperature superconducting (LTS) materials and high field magnets in Nuclear Magnetic Resonance (NMR) and medical Magnetic Resonance Imaging (MRI). NMR spectrometers are used in biology, chemistry and pharmacy to study the structure, the interaction and the kinetics of complex molecules. The expressed need for research spectrometers with the highest possible resolution represents today one of the drives for the development of the high-temperature superconductor (HTS) technology. In fact, in addition to their superior properties at relatively higher temperatures, HTS materials also come along with significantly expanded magnetic field capabilities. Only few months ago, Bruker announced the first ever high-resolution NMR spectra at 1.1 GHz – corresponding at 25.9 T – with a novel HTS/LTS hybrid superconducting magnet [1]: this result belongs also to Switzerland as Bruker BioSpin, one of the industrial members of the MaNEP association, develops and produces its NMR systems in Fällanden, near Zürich.
In the MRI systems, the field homogeneity and stability provided by superconducting magnets are essential to achieve the resolution, precision and speed required for clinical imaging. Commercial MRI systems operate at a magnetic field between 0.5 and 7 T, but ultra-high field devices, up to 11.7 T, are being developed to increase the spatial and temporal resolution of the images with the ambitious goal of decoding the functioning of our brain [2].
Superconductivity and Big Science
Superconductivity is a core technology that has fueled the progress in high-energy physics accelerators and in thermonuclear fusion reactors. The Large Hadron Collider (LHC) at CERN uses more than a thousand superconducting dipoles and quadrupoles, corresponding to about 1200 tons of Nb-Ti wires cooled at 1.9 K. With the discovery of the Higgs boson, LHC has provided further validation to the Standard Model of particle physics.
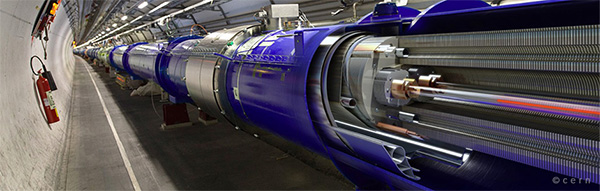
However, many outstanding questions about our understanding of the universe, such as the nature of dark matter or the origin of the observed matter-antimatter asymmetry, are still unanswered and call for particle accelerators at the energy frontier. The Future Circular Collider (FCC) study driven by CERN aims to shed light on these questions at the most fundamental level. The goal of increasing the potential to discover new physics is driving the FCC community to conceive a 100 TeV center-of-mass proton-proton collider, i.e. more than 7 times the design energy of LHC, in a new tunnel of 100 km circumference in the Geneva area. A dipole field of 16 T is necessary to reach the energy target and this is well beyond the limits of Nb-Ti.
CERN and several high-energy physics laboratories in Europe, Asia and North America engage in an intense R&D effort to develop such magnets. The Swiss State Secretariat for Education, Research and Innovation (SERI), together with the ETH Board provide strong financial support to the recently established Swiss collaboration on Accelerator Research and Technology (CHART). It unites CERN, EPFL, ETHZ, PSI and University of Geneva in an effort to further the development of future CERN projects like FCC. One of the main goals of CHART is the development of the 16 Tesla prototype magnet.
Today the prime candidate for the development of the FCC magnets is Nb3Sn, but the critical current performance of state-of-the-art industrial wires is still not sufficient. The record critical current density of Nb3Sn wires has plateaued for more than a decade and many laboratories around the world took up the challenge to lift the wire performance out of stagnation in view of the FCC. The Group of Applied Superconductivity at the University of Geneva is one of them: we are working on the implementation of an innovative approach to refine the grain size of Nb3Sn and, thus, raise the critical current density – the two quantities are inversely proportional – in a wire fabrication process scalable at industrial level. Industrialization of the process is a key parameter as FCC will require superconducting materials in massive quantities – an estimate of 9’000 tons of Nb3Sn! It is worth mentioning that Geneva is one of the very few universities hosting advanced facilities for the development of superconducting wires, thanks to René Flükiger who installed the laboratory at the end of the ’90.
CERN has also plans for upgrading LHC on the medium-short term. The so-called High Luminosity LHC (HL-LHC), which is due to come into operation in 2026 aims to increase the luminosity, i.e. the number of useful interactions, by a factor 5 with respect to the nominal LHC value. The main ingredient of this upgrade is the replacement of the actual quadrupoles in the interacting region with high-field Nb3Sn magnets. HL-LHC will also bring a novel superconducting system for feeding the magnets with high-current MgB2 cables operating at 25 K in helium gas. The present LHC configuration, employs conventional copper cables, with power converters and current leads both located in underground areas. However, all equipment in the tunnel is exposed to significant levels of radiation. The new electrical layout of HL-LHC envisages the location of the power converters away from the tunnel to avoid the radiation damage of these devices and to ease the access of personnel for maintenance. The line in MgB2 will be more compact and lighter than its copper equivalent, and cryogenically more efficient than a classical LTS link. Following a synergetic R&D development between CERN and industries, the team led by Amalia Ballarino has recently tested successfully a 60-metre-long prototype line that transported 40 kA cooled at a temperature of 25 K. This test of the line represents an important milestone in view of its future implementation in the machine, which will need more than 1000 km of MgB2 wire to carry up to 100 kA over lengths of about 150 m. The project has also caught the attention of the outside world. Companies are using the work done at CERN to study the possibility of using similar transmission lines (at high voltage), instead of conventional systems, to transport electricity and power over long distances.
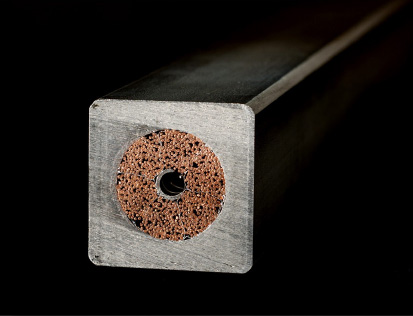
Particle accelerators are used today not only for the advancement of pure knowledge. In the hadron therapy for cancer treatment [3], the tumor is bombarded with a beam of protons or light ions from a particle accelerator. The innovative treatment method is a result of pioneering work at Paul Scherrer Institute (PSI) over the past 30 years and is used for patient treatment there since end of 1996. It has established itself over the past 10 years as the beam delivery and irradiation standard for most of the proton therapy facilities worldwide. More than 60 proton therapy facilities worldwide are using the PSI technology today. Nevertheless the large size and prohibitive cost of such installations severely limit the number of patients that can benefit from this precise method of eradicating cancer cells. The introduction of high-field superconducting magnets is a crucial step forward in this area, reducing the size of different machine components and thus overcoming the economic factors hindering the large diffusion of this technology. In 2001 PSI installed a dedicated compact superconducting 250 MeV proton cyclotron, developed in close collaboration with industry. Presently CERN and PSI are working on innovative designs of compact high field superconducting Gantries to further reduce the size and weight of these facilities.
Superconductors represent also the enabling factor for the International Thermonuclear Experimental Reactor (ITER). The objective of ITER is to demonstrate the technological feasibility of nuclear fusion for energy production. Superconducting cables based on Nb3Sn will be used for generating the high magnetic fields (up to 12 T) needed to confine and shape the high temperature plasma where nuclear fusion takes place. They will operate at currents of several tens of kA and exposed to repeated cycles of axial and transverse loading due to the Lorentz forces. The superconducting wires used for ITER were produced and cabled all around the world (US, Russia, Korea, China, Germany and Italy) but they have all been tested and qualified for use at the Swiss Plasma Center of EPFL, in the SULTAN facility situated at PSI. The first plasma of ITER is scheduled for December 2025 and this will represent an important milestone towards the next-stage device: the Demonstration Power Plant, or DEMO, for the large-scale production of electric power. DEMO will follow ITER around 2050 but a number of other initiatives are being launched by research institutions and private companies around the world (Commonwealth Fusion Systems in US, Tokamak Energy in UK, …). Outstanding technological and physics integration issues still need to be tackled, but Switzerland is already confirming its leading role in the superconducting technologies.
Power generation and distribution: the role of superconductors
Profound changes affecting the electric power sector, like renewables sources and distributed generation, offer an unprecedented opportunity to transform the electric power infrastructure. HTS are potentially key in the suite of technologies that can help facilitate grid modernization and increase energy security. Powerful new superconducting generators, high-capacity cables and fault current limiters are among the solutions that will enhance the efficiency and reliability of electricity generation, transport and distribution. It is worth emphasizing here that the NCCR MaNEP pioneered the studies on fault current limiters with the activities of Bertrand Dutoit at EPFL, Michel Decroux at University of Geneva and Markus Abplanalp at ABB.
In the generator application, superconductors provide technical solutions to upscale the power generation and reduce the weight of wind turbines, which will also lower the production price of the electricity from renewable sources. The partners of the European project ECOSWING recently tested the first superconducting wind turbine connected to the electricity grid in Thyborøn, Denmark [4]. Many Danish households could claim to be “powered by superconductivity”!
Among all HTS power applications, cables are probably the most experienced. One of the main advantage resulting from the use of HTS cables is their high power density. In congested urban areas, expanding the capacity of an underground power line can involve digging up streets and can be expensive and disruptive. Because superconductors can carry orders of magnitude more current than conventional conductors of the same cross-section, a convenient use for HTS cables is their installation as retrofit cables in some existing paths where the conventional copper cables are operating at their limits. The AMPACITY project represents a remarkable example [5]: in the city of Essen, Germany, a 10 kV-2.3kA superconducting cable has been integrated in the electric grid of the downtown since 2014. The cable has made it possible to simplify the architecture of the distribution network without giving up redundancy, while adding resilience thanks to a fault current limiter.
Sustainable mobility: the dream of a superconducting airliner
The use of superconductors also offers promise of innovations in the field of mobility. The better-known example is the Central Japan Railway project for the construction of the Chuo Shinkansen Maglev Line, a magnetically levitated high-speed train between Tokyo and Nagoya, covering the distance of about 500 km between the two cities in one hour. However, the greatest advantages are coming with HTS-based motors: thanks to the strong reduction of the energy losses and the intense magnetic fields, superconductors can make propulsion systems smaller, lighter and more powerful. Sustainability in the aviation industry calls for aircraft that are significantly quieter and more fuel efficient than today’s fleet. Achieving this will require revolutionary new concepts and, in particular, electric propulsion. HTS machines offer a viable path that would allow integration of electric propulsion within the very stringent weight and volume constraints imposed by an airborne application. These benefits have already attracted the interest from Airbus, Rolls-Royce, Siemens and other big players in the field [6]. One suggestive option that is being explored is the use of liquid hydrogen as a fuel, either for turbines or fuel cells. This would allow cooling the superconducting machines for free: an excellent synergy that fits perfectly in the vision of a future sustainable society where hydrogen is used as a low carbon fuel, energy storage and long distance transport of energy.
Conclusions
The progresses witnessed in the last year in the applications of superconductors illustrate the close synergetic relationship between evolution of the material understanding and the advancement in the technology. This success was possible thanks to a virtuous circle encompassing fundamental science, applied research and industrial production. Switzerland is a very special example of this virtuous circle: thanks to initiatives like MaNEP and CHART, universities, research centers and industries work closely together, also facilitated by the geographical proximity of laboratories, and this allows our research to play a leading role on the international scene.
[1] Bruker Corp. company presentation, 7 January 2019, //s22.q4cdn.com/617463959/files/doc_presentations/2019/01/JPM19HCC-BRKR-FINAL-04Jan19.pdf
[2] NeuroSpin, //neurospin.org
[3] //www.psi.ch/en/protontherapy/center-for-proton-therapy-cpt
[4] h2020 project ecoswing, //ecoswing.eu/
[6] h2020 project ASuMED, //asumed.oswald.de/
(Photo credit: CERN)