Novel Materials Research Enabled by SwissFEL
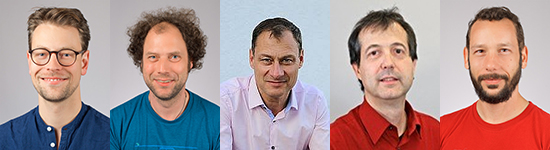
By Simon Gerber, Henrik Lemke, Luc Patthey, Urs Staub and Cristian Svetina, Paul Scherrer Institut
The X-ray free-electron laser SwissFEL [1] is PSI’s latest large-scale facility, serves several research areas and is complementary to the other accelerator-based photon source, the Swiss Light Source (SLS) synchrotron [2]. For condensed matter research, SwissFEL operates the Bernina endstation [3] at the hard X-ray Aramis beamline. In addition, the Cristallina endstation [4] is under construction, serving in parts also condensed matter research, as well as, the Furka endstation [5] at the soft X-ray Athos beamline. Here we provide a short overview of the status of the current instrumentation, describe two experiments from the operational Bernina endstation, and close with an outlook. An overview of future directions in X-ray science is found in the recently published Photon Science Roadmap [6] composed by the Swiss Society for Photon Science.
Novel functionalities of materials properties are based on the interplay between properties of the atoms or molecules in a crystal, such as electronic and magnetic exchange interactions. Changes of these properties can occur either through phase transitions or continuously depend on, e.g. pressure, temperature, electric and magnetic fields, which can define or generate macroscopic magnetic and electronic quantum states. An understanding of the mechanisms which govern the transition between states with different novel properties is typically complex, especially when triggered via an out-of-equilibrium excitation. This is often related to a large number of degrees of freedom which defines the states, and is further complicated by complexity of theoretically describing out-of-equilibrium processes.
Valuable insight into functionalities can be gained by collecting ultrafast snapshots of the properties during a state change. These snapshots can then reveal the pathway taken in a multidimensional energy potential. It is, thereby, possible to temporally resolve even electronic changes via ultrafast laser excitation. Facilities like SwissFEL provide new insights into structures, electronic and magnetic states of matter, which is complementary to the information obtained by ultrafast optics obtained in the last decades. Most importantly, one can now obtain direct and quantifiable microscopic information. High-energy X-rays can not only resolve atomic length scale structures from the crystal lattice, but also electronic and magnetic states by tuning the photon energy to the X-ray absorption edges of the atoms of interest.
Namely, the Bernina endstation [3] already operates in a photon energy range from 2 to 12 keV, giving access to a large fraction of X-ray absorption edges of 3d, 4d and 5d transition metal, as well as 4f and 5f rare-earth ions. This allows for ultrafast spectroscopic probes and resonant diffraction, providing insight into the ultrafast dynamics of electronic and magnetic structures and their transformative changes. Two exchangeable diffractometers facilitate different experimental geometries and analysis of the diffracted photons in terms of polarization and energy, as well as flexible usage of sample environments, e.g. cryostats or cryomagnets. A 20 mJ Ti:sapphire laser system is used to generate ultrafast light pulses ranging from UV to mid-IR and THz frequencies. Those ‘pump’ pulses are used to excite materials at specific electronic and vibrational transitions which in turn allow to study particular excitation mechanisms of a transition.
Cristallina [4] is the third experimental station of the Aramis hard X-ray beamline. It serves both quantum science (Cristallina-Q) and structural biology (Cristallina-MX) by enabling imaging of quantum many-body states under extreme conditions as well as serial femtosecond protein crystallography, respectively. The beamline is optimized to deliver highly focused, sub-femtosecond X-ray pulses, which is uniquely complemented by integrated offline preparation areas. The two baseline Cristallina-Q experimental setups will be installed on two flexibly movable diffractometers and provide (pulsed and static) high-magnetic-field – low-temperature capabilities. Cristallina-Q is realized in close collaboration with the group of Johan Chang and co-funded by UZH. Commissioning of the beamline and the scientific instruments is planned for 2022.
In addition, the soft X-ray beamline Athos is currently under commissioning and will be ready for user experiment in second half of 2021. This beamline will deliver X-ray free-electron laser (FEL) radiation in the photon energy range between 250 and 1900 eV [7]. Owing to two-bunch operation and a fast bunch separation system, the Athos beamline will be operated up to the full SwissFEL repetition rate of 100 Hz without disturbing the existing Aramis hard X-ray branch [8]. Athos includes a novel layout of alternating magnetic chicanes and short undulator segments, dubbed CHIC. Together with the APPLE X undulator architecture [9], the Athos branch can be operated in a wide range of modes producing FEL beams with unique characteristics ranging from attosecond pulse length, high-power, high or narrow bandwidth, full polarization control, transverse gradient to two-color modes. Further space has been reserved for upgrades including modulators and an external seeding laser for better timing control. The optical transport line distributing the FEL beam to the experimental stations was specifically designed to accept this large range of beam parameters [10]. Currently two experimental stations, one for quantum materials research (Furka), as well as one for atomic, molecular and optical physics, chemical sciences and ultrafast single-particle imaging (Maloja), are being laid out such that they can optimally profit from the unique soft X-ray pulses.
The Furka experimental station (see Fig. 1) is dedicated to time-resolved resonant inelastic X-ray scattering (tr-RIXS) and (resonant) diffraction (tr-XRD) to study ultrafast dynamics in quantum matter. We recall that many properties of quantum materials originate from couplings between charge, orbital, spin and lattice degrees of freedom. These couplings lead to cross-correlations among different physical observables, which then can be tuned towards application of the emergent functionality. Mott transitions, high-temperature superconductivity, colossal magnetoresistance, giant magneto-electric effect and topological insulators are just a few examples of the remarkable properties that arise from the collective behavior of the different degrees of freedom. Ultrafast techniques, especially femtosecond spectroscopy or diffraction, enabled by the advent of Athos, now create opportunities for direct measurements of the coupling strength between the different degrees of freedom with unprecedented precision at temperatures even below 10 Kelvin. In such femtosecond pump–probe experiments, selected excitation mechanism are used to probe, e.g. electronic, magnetic and structural dynamics and low-energy quasiparticle excitations, as well as correlations and fluctuations in non-equilibrium systems.
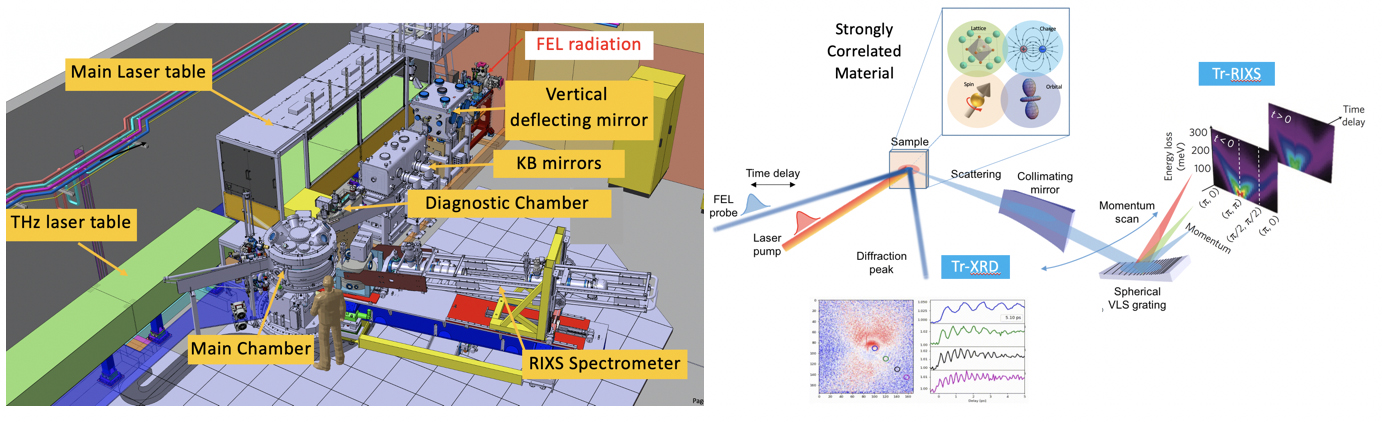
In the following, two examples of successful SwissFEL experiments are described. In an early experiment at the Bernina endstation, a combined X-ray absorption and diffraction study focused on the question of electron delocalization in a correlated metal [12]. In general, ultrafast electron delocalization induced by a femtosecond laser pulse is a well-known process in which electrons are ejected from atoms or ions within the laser pulse duration, leading to an increase of “delocalized” electrons. However, the speed of electron localization except from direct electronic screening effects, is not well understood nor well studied. In particular, localization out of an electron gas, i.e. the capture of an electron by an ion, is unknown. In this experiment, it has been demonstrated by means of pump-probe X-ray techniques with energies around the Eu L3 absorption edge that the electron localization process in the intermetallic EuNi2(Si0.21Ge0.79)2 occurs within a few-hundred femtoseconds after the optical (800 nm) excitation. Spectroscopy and diffraction data collected simultaneously at a temperature of 90 Kelvin and for various laser fluences (see Fig. 2) show that the localization dynamics process is much faster than the thermal lattice expansion along the crystallographic c-direction which occurs on the picosecond timescale. Nevertheless, the latter process is still much slower than pure electronic effects such as screening. The sub-picosecond timescale of the localization dynamics indicates a phonon driven origin. In addition, comparing the laser fluence dependence of the electronic response with that found in other intermediate 4f valence materials, suggest that the electron localization process observed in this Eu-based correlated metal is mainly related to changes in the 4f hybridization. The observed ultrafast electron localization process sparks fundamental questions for our understanding of electron correlations and their coupling to the lattice, in particular concerning valence transitions in correlated 4f electron metals.
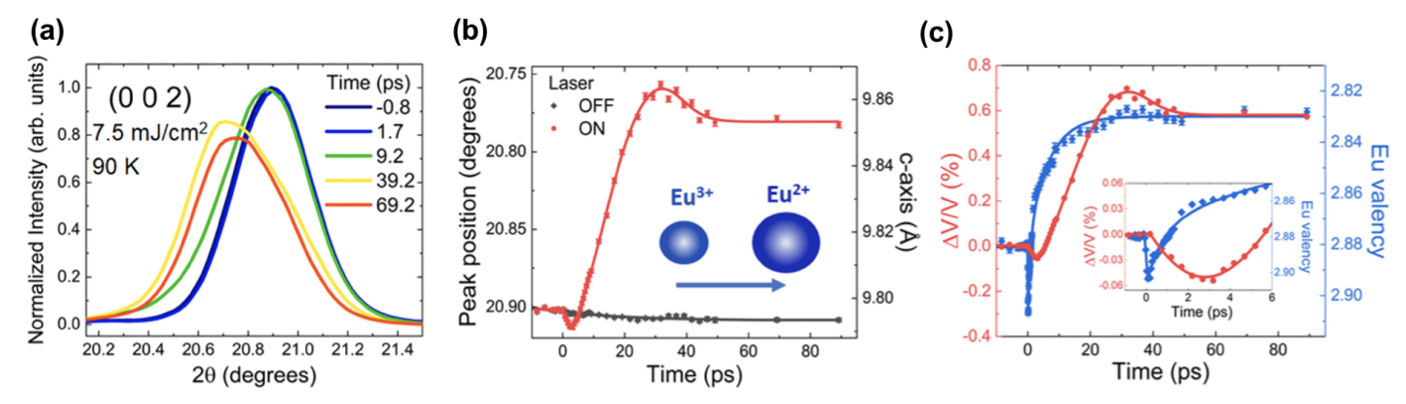
A second successful SwissFEL experiment concerns nonlinear X-ray science. Such techniques are based on the nonlinear response of a medium after excitation from electric fields. In particular, four-wave mixing (FWM) can access the third-order susceptibility by employing three independent laser pulses with specific wavelengths, polarizations, wave-vectors and controllable time delay crossing at the sample. A special case of FWM is time-resolved transient grating (TG) spectroscopy in which two excitation pulses have the same wavelength and arrival time. This configuration transiently excites the sample with a spatial periodic modulation, corresponding to a specific momentum transfer. The time dependent change of the index of refraction can then be measured via scattering of a third, delayed laser pulse. The technique provides “background-free” detection covering up to 15 orders of magnitude in time. Optical TG is used since decades in condensed matter research and has been applied for detection of e.g. electron-phonon coupling strengths, charge, spin, phonon, density and mass transport, as well as charge and spin density waves. However, optical wavelength accessed only very limited momentum transfer and no element selectivity is possible. Indeed, TG spectroscopy has been extended to the EUV regime some years ago, allowing to reach tens of nanometer excitation grating and M shell resonances [13]. However, the short attenuation length and large recombination angles, as well as the time resolution, present stringent limitation for application to quantum materials. In a recent SwissFEL experiment, the step to extend TG into the X-ray regime has been accomplished by employing a diamond phase grating and taking advantage of the Talbot effect [14]. At the Bernina endstation, excitation gratings were generated on bismuth germanate at 7.1 keV and a 400 nm optical probe laser was used to detect the change in the material’s response (see Fig. 3) [15]. This achievement opens new possibilities to explore resonant pump excitations, both at the surface and in the bulk, with various applications in condensed matter physics. The final step will be to implement an all X-ray TG, allowing to overcome the limitation of optical probe by adding element selectivity in the probe, as well as sub-femtosecond temporal and sub-nanometer special resolution. Applications of all X-ray TG experiments are, but not limited to, investigations of electron-electron and electron-phonon coupling strengths, charge, spin and heat transport at the nanoscale, ultrafast demagnetization dynamics, generation and detection of charge and spin density waves and skyrmions, as well as the study of collective dynamics of disordered systems.
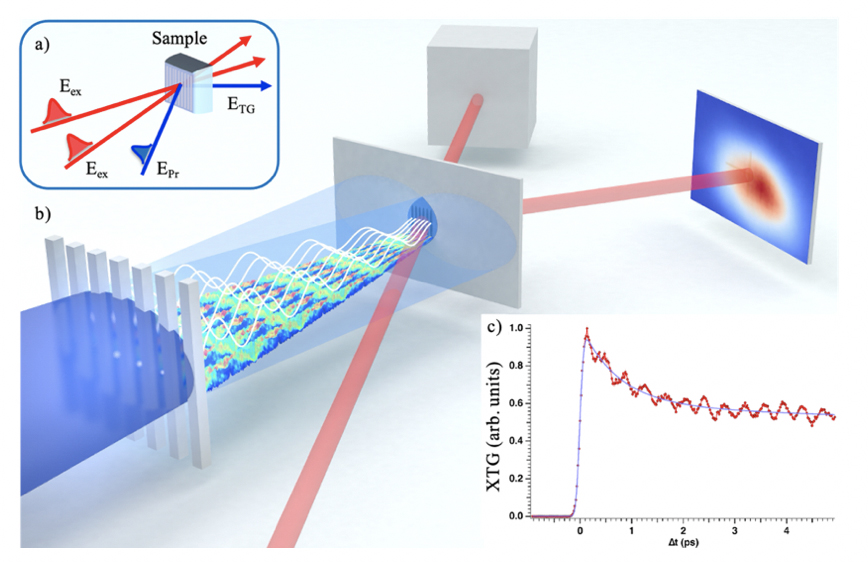
We close by elaborating on possible future directions of nonequilibrium quantum matter experiments at SwissFEL. Generally, SwissFEL users and staff will continue to push for higher temporal resolution by further optimizing the synchronization amongst the optical pump and X-ray probe pulses, as well as utilizing ultrashort (sub-femtosecond) pulses, now available both in the soft and hard X-ray regime. New opportunities in condensed matter research will also be facilitated when the third SwissFEL beamline Porthos, as recently brought forward in the Photon Science Roadmap of the Swiss Academy of Sciences [6], gets financed. The goal is pursued to transfer the unique developments achieved in the soft X-ray regime at the Athos beamline, e.g. CHIC operation modes and APPLE X undulators [7], to hard X-rays. For example, full polarization and bandwidth control over the X-ray pulses will enable single-shot pump-probe X-ray magnetic circular dichroism and resonant tr-XRD.
Finally, both at Athos [16] and also Porthos [6], it has also been suggested to combine such schemes with self-seeding. Namely, selective electron bunch degradation and transverse beam shaping in the accelerator, combined with a self-seeded photon emission, can yield phase-locked pulse pairs or trains [16]. Equivalently, a fixed phase relation amongst X-ray FEL pulses can also be achieved via seeding by an optical laser, as being implemented at Athos as a part of the ERC Synergy project HERO [17]. Trains of phase-locked pulses hold the potential to extend high-resolution frequency comb spectroscopy from the optical to the X-ray regime. In particular, phase-locked pulse pairs enable time-domain X-ray Ramsey interferometry. Such experiments will build on quantum interference of two photon fields with a variable time delay and fixed relative phase relation, translating into a radiation power spectrum with tunable modulation. One particular application is the X-ray analog of the ubiquitous Fourier transform infrared (FTIR) spectrometer, e.g. for efficient determination of electronic absorption line widths of core-hole lifetimes and their impact on valence electron states. In addition, resonant and non-resonant inelastic scattering can be achieved by tuning the radiation power spectrum modulation (instead of scanning a monochromator), e.g. to establish dispersion relations of low-energy excitations or auto-correlation measurements of speckles. Ultimately, also tuning of the phase difference and relative amplitude of pulses is possible, paving the way towards coherent control and read-out of quantum states using SwissFEL’s ultrafast X-rays pulses.
[1] www.psi.ch/swissfel
[2] www.psi.ch/sls
[3] www.psi.ch/swissfel/bernina
[4] www.psi.ch/swissfel/cristallina
[5] www.psi.ch/swissfel/furka
[6] R. Abela et al., Photon science roadmap for research infrastructures 2025–2028, Swiss Acad. Rep. 16, 5 (2021).
[7] R. Abela et al., The SwissFEL soft X-ray free-electron laser beamline: Athos, J. Synchrotron Rad. 26, 1073 (2019).
[8] C. J. Milne et al., SwissFEL: The Swiss X-ray free electron laser, Appl. Sci. 7, 720 (2017).
[9] X. Liang et al., Analysis of the first magnetic results of the PSI APPLE X undulators in elliptical polarization, Nucl. Instr. and Meth. Phys. Research A 987, 164741 (2021).
[10] R. Follath, U. Flechsig, U.H. Wagner & L. Patthey, Optical design of the Athos beamlines at SwissFEL, AIP Conf. Procs. 2054, 060024 (2019).
[11] M. P. M. Dean et al., Ultrafast energy- and momentum-resolved dynamics of magnetic correlations in the photo-doped Mott insulator Sr2IrO4, Nat. Mat. 15, 601 (2016).
[12] J. R. L. Mardegan et al., Ultrafast electron localization in the EuNi2(Si0.21Ge0.79)2 correlated metal, arXiv:2002.12214.[13] F. Bencivenga et al., Four-wave mixing experiments with extreme ultraviolet transient gratings. Nature 520, 205 (2015).
[14] C. Svetina et al., Towards X-ray transient grating spectroscopy. Opt. Lett. 44, 574 (2019).
[15] J. R. Rouxel et al., Hard X-ray transient grating spectroscopy on bismuth germanate, Nat. Photon., 10.1038/s41566-021-00797-9 (2021).
[16] S. Reiche et al., Towards the perfect X-ray beam splitter, arXiv:2010.00230.
[17] //synergyhero.org